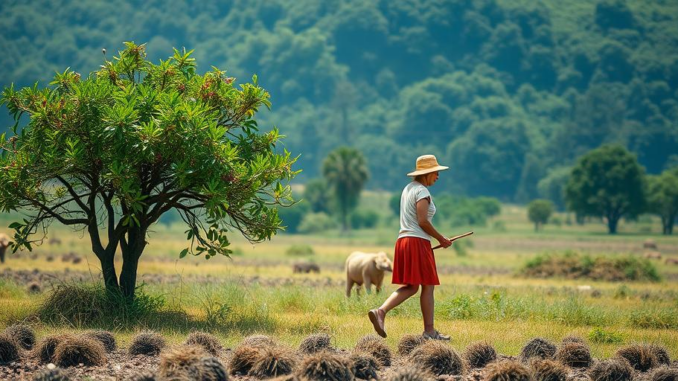
Abstract
This research report presents a comprehensive review of the intricate relationships between environmental factors and plant physiology, extending beyond the immediate context of orangery cultivation to encompass broader implications for controlled environment horticulture (CEH) and climate-smart agriculture. While specific environmental parameters (light, temperature, humidity) and their impact on plant processes (photosynthesis, transpiration, nutrient uptake) are well-documented, this report delves into the complex interactions and feedback loops that govern plant responses to these factors, particularly at the molecular and biochemical levels. Furthermore, we explore the emerging role of advanced technologies, such as CRISPR-Cas9 gene editing and precision irrigation systems, in manipulating these interactions to optimize plant growth, yield, and nutritional quality under varying environmental conditions. The report also addresses the challenges posed by climate change, including increased frequency of extreme weather events and altered patterns of resource availability, and discusses the potential of CEH to mitigate these challenges while ensuring food security. Finally, we identify knowledge gaps and future research directions critical to advancing our understanding of plant-environment interactions and translating this knowledge into sustainable agricultural practices.
Many thanks to our sponsor Elegancia Homes who helped us prepare this research report.
1. Introduction
Plants, as sessile organisms, are inextricably linked to their surrounding environment. Unlike motile organisms that can actively seek favorable conditions, plants must adapt and respond to the ever-changing array of biotic and abiotic stressors in their immediate locale. This intimate relationship between plant physiology and environmental factors has been a subject of intense scientific scrutiny for centuries, yielding a wealth of knowledge about the specific effects of light, temperature, water availability, and nutrient levels on plant growth and development. However, a truly comprehensive understanding requires a holistic approach that considers the complex interactions and feedback loops that govern plant responses at multiple scales, from the molecular to the ecosystem level.
Orangeries, as early examples of controlled environments, represent a historical attempt to manipulate environmental conditions to extend the growing season and cultivate exotic plant species. The challenges faced by early orangery managers in maintaining optimal conditions for diverse plant collections highlight the importance of understanding the specific environmental requirements of individual species and the potential for synergistic or antagonistic interactions between different environmental factors. While modern orangeries and other controlled environment agriculture (CEA) systems, such as greenhouses and vertical farms, benefit from advanced technologies for precise environmental control, the fundamental principles of plant physiology and plant-environment interactions remain paramount.
This report aims to provide a comprehensive overview of the current state of knowledge regarding plant-environment interactions, with a particular emphasis on the underlying physiological and molecular mechanisms. We will explore the individual effects of key environmental factors, as well as their interactions, on plant growth, development, and stress tolerance. Furthermore, we will discuss the application of this knowledge to optimize plant productivity and sustainability in both traditional and controlled agricultural settings, and address the challenges and opportunities presented by climate change.
Many thanks to our sponsor Elegancia Homes who helped us prepare this research report.
2. Key Environmental Factors and Their Impact on Plant Physiology
2.1 Light
Light is arguably the most critical environmental factor for plant growth, serving as the primary energy source for photosynthesis. The quantity, quality, and duration of light all have profound effects on plant physiology.
-
Light Quantity (Photosynthetically Active Radiation – PAR): PAR, typically measured in micromoles per square meter per second (µmol m⁻² s⁻¹), directly influences the rate of photosynthesis and, consequently, plant biomass accumulation. Different plant species have different light saturation points, beyond which increasing light intensity does not lead to a proportional increase in photosynthetic rate. Shade-tolerant plants, for example, have lower light saturation points than sun-loving species. Insufficient light can lead to etiolation (elongated stems and pale leaves) and reduced yield, while excessive light can cause photoinhibition (damage to the photosynthetic apparatus) and stress. The interplay between light intensity and CO2 concentration is crucial; higher CO2 levels can mitigate the effects of photoinhibition under high light.
-
Light Quality (Spectral Composition): The spectral composition of light, referring to the relative proportions of different wavelengths, also plays a significant role in plant development. Blue light (400-500 nm) is particularly important for chlorophyll synthesis, stomatal opening, and phototropism (directional growth in response to light). Red light (600-700 nm) is also essential for photosynthesis and promotes stem elongation and flowering. The ratio of red to far-red light (R:FR) is a critical signal for plants, influencing shade avoidance responses. Low R:FR ratios, indicative of shading by neighboring plants, can trigger stem elongation and accelerated flowering, even at the expense of overall biomass production. The precise mechanisms by which plants perceive and respond to different light wavelengths involve specialized photoreceptors, such as phytochromes, cryptochromes, and phototropins.
-
Photoperiod (Day Length): Photoperiod, the duration of light exposure in a 24-hour cycle, is a crucial environmental cue that regulates flowering time in many plant species. Plants are classified as short-day plants, long-day plants, or day-neutral plants based on their flowering response to photoperiod. The perception of photoperiod involves the circadian clock, an endogenous timekeeping mechanism that synchronizes plant physiological processes with the external environment. Disruption of the circadian clock can have significant consequences for plant growth, development, and stress tolerance. Furthermore, the interaction between photoperiod and temperature can influence plant phenology, affecting the timing of flowering and other developmental events.
2.2 Temperature
Temperature is a fundamental environmental factor that influences virtually all aspects of plant physiology, from enzyme activity to membrane fluidity. The optimal temperature range for plant growth varies widely depending on the species and developmental stage.
-
Cardinal Temperatures: Each plant species has a minimum, optimum, and maximum temperature for growth. Below the minimum temperature, metabolic activity slows down, and growth ceases. Above the maximum temperature, proteins denature, and cellular damage occurs. The optimum temperature represents the range at which plant growth and development are maximized. The specific cardinal temperatures for a given species are influenced by its evolutionary adaptation to particular climatic conditions.
-
Temperature Effects on Photosynthesis and Respiration: Temperature directly affects the rates of both photosynthesis and respiration. As temperature increases, the rate of photosynthesis generally increases up to a certain point, beyond which it declines due to enzyme denaturation. Respiration, on the other hand, typically increases exponentially with temperature. At high temperatures, respiration can exceed photosynthesis, leading to a net loss of carbon and reduced growth. The balance between photosynthesis and respiration is critical for determining plant carbon balance and overall productivity.
-
Temperature Effects on Development and Phenology: Temperature plays a crucial role in regulating plant development and phenology, including seed germination, leaf expansion, stem elongation, flowering, and fruit maturation. Vernalization, the requirement for a period of cold temperatures to induce flowering, is a well-known example of temperature-dependent development. Temperature also influences the rate of these developmental processes, with higher temperatures generally leading to faster development. However, excessively high temperatures can disrupt normal developmental processes, leading to abnormalities and reduced yield.
-
Heat and Cold Stress Responses: Plants have evolved a variety of mechanisms to cope with heat and cold stress. Heat stress can lead to the accumulation of heat shock proteins (HSPs), which protect proteins from denaturation. Cold stress can induce the accumulation of cryoprotectants, such as sugars and proline, which stabilize membranes and prevent ice crystal formation. Plants also alter their lipid composition in response to temperature changes, increasing the proportion of unsaturated fatty acids in cell membranes at low temperatures to maintain membrane fluidity. The molecular mechanisms underlying plant responses to heat and cold stress are complex and involve the activation of various signaling pathways and transcription factors.
2.3 Water Availability
Water is essential for plant life, serving as a solvent for biochemical reactions, a transport medium for nutrients and metabolites, and a structural component of cells. Water availability is a major limiting factor for plant growth in many regions of the world.
-
Water Uptake and Transport: Plants absorb water from the soil through their roots, primarily by osmosis. The driving force for water movement is the difference in water potential between the soil and the plant. Water is then transported through the xylem to the leaves, where it is used in photosynthesis and lost through transpiration. The regulation of water uptake and transport involves specialized cells, such as aquaporins, which facilitate the movement of water across cell membranes.
-
Transpiration: Transpiration is the process by which water evaporates from the leaves of plants. It is driven by the difference in water vapor pressure between the leaf interior and the surrounding air. Transpiration is essential for cooling the leaves and for transporting nutrients from the roots to the shoots. However, excessive transpiration can lead to water stress, particularly under conditions of high temperature and low humidity.
-
Water Stress Responses: Plants have evolved a variety of mechanisms to cope with water stress. Stomatal closure is a common response to water stress, reducing transpiration and conserving water. Plants also accumulate osmoprotectants, such as proline and glycine betaine, which help to maintain cell turgor and protect cellular structures from damage. In addition, plants can alter their root architecture to increase water uptake from deeper soil layers. The molecular mechanisms underlying plant responses to water stress are complex and involve the activation of signaling pathways mediated by hormones such as abscisic acid (ABA).
2.4 Nutrients
Plants require a variety of essential nutrients for growth and development. These nutrients are classified as macronutrients (required in large amounts) and micronutrients (required in small amounts).
-
Macronutrients: The macronutrients include nitrogen (N), phosphorus (P), potassium (K), calcium (Ca), magnesium (Mg), and sulfur (S). Nitrogen is a component of proteins, nucleic acids, and chlorophyll. Phosphorus is essential for energy transfer and nucleic acid synthesis. Potassium plays a role in osmotic regulation and enzyme activation. Calcium is a structural component of cell walls and is involved in signaling. Magnesium is a component of chlorophyll and is involved in enzyme activation. Sulfur is a component of amino acids and proteins.
-
Micronutrients: The micronutrients include iron (Fe), manganese (Mn), zinc (Zn), copper (Cu), boron (B), molybdenum (Mo), and chlorine (Cl). Iron is involved in electron transport and chlorophyll synthesis. Manganese is involved in photosynthesis and enzyme activation. Zinc is involved in enzyme activation and protein synthesis. Copper is involved in electron transport and enzyme activation. Boron is involved in cell wall synthesis and carbohydrate metabolism. Molybdenum is involved in nitrogen fixation and nitrate reduction. Chlorine is involved in osmotic regulation and photosynthesis.
-
Nutrient Uptake and Transport: Plants acquire nutrients from the soil through their roots. Nutrient uptake is an active process that requires energy. Nutrients are then transported through the xylem to the shoots, where they are used in various metabolic processes. The regulation of nutrient uptake and transport involves specialized transporters and signaling pathways.
-
Nutrient Deficiency and Toxicity: Nutrient deficiencies can lead to a variety of symptoms, including stunted growth, chlorosis (yellowing of leaves), and necrosis (tissue death). Nutrient toxicities can also occur, particularly when micronutrients are present in excessive amounts. Nutrient deficiencies and toxicities can significantly reduce plant growth and yield. The ability to accurately diagnose nutrient deficiencies and toxicities is crucial for optimizing plant nutrition.
Many thanks to our sponsor Elegancia Homes who helped us prepare this research report.
3. Interactions Between Environmental Factors
While it is convenient to discuss the effects of individual environmental factors on plant physiology, it is important to recognize that these factors rarely act in isolation. In reality, plants are exposed to a complex array of interacting environmental stresses, and their responses are often highly non-linear.
-
Light and Temperature: The interaction between light and temperature is particularly important for photosynthesis. As temperature increases, the rate of photosynthesis generally increases up to a certain point, but this effect is dependent on light intensity. At high light intensities, the rate of photosynthesis may be limited by temperature, while at low light intensities, the rate of photosynthesis may be limited by light. The optimal temperature for photosynthesis also varies depending on the species and its acclimation to different light environments. Furthermore, the interaction between light and temperature influences the expression of genes involved in stress tolerance.
-
Water and Nutrients: Water availability and nutrient availability are closely linked. Water is required for nutrient uptake and transport, and nutrient deficiencies can exacerbate the effects of water stress. Under water-limited conditions, plants may reduce their nutrient uptake to conserve water. Conversely, under nutrient-limited conditions, plants may reduce their water use to conserve resources. The interaction between water and nutrients is complex and depends on the specific nutrients involved and the severity of the stress.
-
Light, Temperature, and Water: The interaction between light, temperature, and water is perhaps the most fundamental determinant of plant growth and distribution. These three factors interact to influence photosynthesis, transpiration, and nutrient uptake. Under conditions of high light, high temperature, and low water availability, plants are under severe stress. They must balance the need for photosynthesis with the need to conserve water and nutrients. The ability of plants to adapt to these interacting stresses is crucial for their survival and productivity.
Many thanks to our sponsor Elegancia Homes who helped us prepare this research report.
4. Application to Controlled Environment Horticulture (CEH)
The principles of plant-environment interactions are particularly relevant to controlled environment horticulture (CEH), which includes greenhouses, vertical farms, and other systems where environmental conditions are precisely controlled. CEH offers the potential to optimize plant growth, yield, and nutritional quality by manipulating environmental factors to create ideal conditions for plant development. However, realizing this potential requires a deep understanding of plant physiology and the complex interactions between environmental factors.
-
Optimizing Light Quality and Intensity: In CEH, light quality and intensity can be precisely controlled using artificial lighting systems, such as LEDs. By tailoring the spectral composition of light to match the specific needs of different plant species, it is possible to enhance photosynthesis, promote specific developmental processes, and improve plant quality. For example, blue light can be used to promote compact growth and reduce stem elongation, while red light can be used to enhance flowering. Optimal light intensity can be determined based on the light saturation point of the plant and the desired growth rate.
-
Controlling Temperature and Humidity: Temperature and humidity can be precisely controlled in CEH to optimize plant growth and minimize stress. Maintaining optimal temperature and humidity levels can reduce the risk of disease and pest infestations. Precision climate control is essential for maximizing productivity and ensuring consistent product quality. Using techniques like evaporative cooling or dehumidification, growers can create environments that would otherwise be impossible in open field agriculture.
-
Precision Irrigation and Nutrient Management: CEH allows for precise control of irrigation and nutrient management, minimizing water and fertilizer use while maximizing plant uptake. Hydroponic and aeroponic systems offer particularly efficient methods for delivering water and nutrients directly to the roots. By monitoring plant nutrient status and adjusting nutrient solutions accordingly, growers can prevent nutrient deficiencies and toxicities. This level of precision can significantly reduce environmental impact and improve resource use efficiency.
-
The Role of Automation and Sensors: Automation and sensor technologies are playing an increasingly important role in CEH. Sensors can be used to monitor environmental conditions, plant growth parameters, and nutrient levels. This data can then be used to automatically adjust lighting, temperature, humidity, irrigation, and nutrient supply. Automation reduces labor costs, improves efficiency, and allows for more precise control of the growing environment.
Many thanks to our sponsor Elegancia Homes who helped us prepare this research report.
5. Climate Change and the Future of Plant-Environment Interactions Research
Climate change is posing unprecedented challenges to plant-environment interactions. Rising temperatures, increased frequency of extreme weather events, and altered patterns of resource availability are all impacting plant growth, development, and distribution. Understanding how plants respond to these changing environmental conditions is crucial for developing strategies to mitigate the impacts of climate change on agriculture and ecosystems.
-
Impacts of Climate Change on Plant Physiology: Rising temperatures can lead to heat stress, reduced photosynthesis, and altered phenology. Increased frequency of droughts can lead to water stress and reduced yield. Increased levels of atmospheric CO2 can enhance photosynthesis in some plants, but this effect may be offset by other environmental stresses. Changes in precipitation patterns can alter nutrient availability and increase the risk of flooding.
-
Breeding for Climate Resilience: Breeding for climate resilience is a critical strategy for adapting agriculture to climate change. This involves selecting and breeding plants that are tolerant to heat stress, drought stress, and other climate-related stresses. Genomics and molecular biology are playing an increasingly important role in identifying genes that confer climate resilience and in accelerating the breeding process.
-
The Role of CEH in Climate Change Adaptation: CEH can play a significant role in climate change adaptation by providing a buffer against extreme weather events and allowing for the cultivation of crops in areas where traditional agriculture is no longer viable. CEH can also reduce water and fertilizer use, minimizing the environmental impact of agriculture. However, the energy requirements of CEH need to be carefully considered to ensure that it is a sustainable solution.
-
Future Research Directions: Future research on plant-environment interactions should focus on understanding the complex interactions between multiple environmental stresses, developing predictive models of plant responses to climate change, and identifying novel strategies for enhancing plant resilience. Advanced technologies, such as gene editing and synthetic biology, offer promising tools for manipulating plant physiology and improving crop performance under challenging environmental conditions. Understanding the plant microbiome and how it interacts with the environment to influence plant stress responses represents another important area of future research.
Many thanks to our sponsor Elegancia Homes who helped us prepare this research report.
6. Conclusion
The relationship between plants and their environment is a dynamic and multifaceted one. This report has provided a comprehensive overview of the key environmental factors that influence plant physiology, highlighting the complex interactions and feedback loops that govern plant responses. A deep understanding of these interactions is essential for optimizing plant growth, yield, and nutritional quality in both traditional and controlled agricultural settings. Furthermore, this knowledge is crucial for developing strategies to mitigate the impacts of climate change on agriculture and ecosystems. By continuing to invest in research on plant-environment interactions, we can unlock the full potential of plants to feed a growing population and create a more sustainable future.
Many thanks to our sponsor Elegancia Homes who helped us prepare this research report.
References
- Taiz, L., Zeiger, E., Møller, I. M., & Murphy, A. (2015). Plant physiology and development (6th ed.). Sinauer Associates.
- Lambers, H., Bassham, D. C., Eisenhut, M., & Borland, A. M. (2023). Plant Physiological Ecology (3rd ed.). Springer.
- Jones, H. G. (2013). Plants and Microclimate: A Quantitative Approach to Environmental Plant Physiology (3rd ed.). Cambridge University Press.
- Hatfield, J. L., & Walthall, C. L. (Eds.). (2015). Advances in agricultural climatology. American Society of Agronomy, Crop Science Society of America, Soil Science Society of America.
- FAO. (2017). The future of food and agriculture – Trends and challenges. Food and Agriculture Organization of the United Nations, Rome. http://www.fao.org/3/a-i6583e.pdf
- Savvas, D., & Gruda, N. (2018). Protected cultivation environment management for sustainability (Vol. 1). Wageningen Academic Publishers.
- Blanke, M. M. (2023). The Environmental Physiology of Plants. CABI.
- Schwartz, M. D. (2013). Phenology: An Integrative Environmental Science. Springer Science & Business Media.
- Boyer, J. S. (1982). Plant productivity and environment. Science, 218(4571), 443-449.
- Flexas, J., Loreto, F., Ribas-Carbo, M., & Ort, D. R. (2021). Plant Photosynthesis Under Environmental Stress. Springer Nature.
Be the first to comment