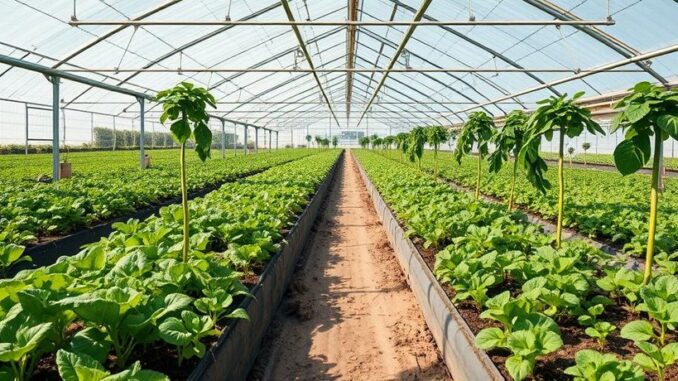
Optimizing Controlled Environment Agriculture: A Comprehensive Analysis of Plant Physiology, Environmental Control, and Sustainable Practices in Orangeries and Similar Structures
Abstract
Controlled Environment Agriculture (CEA), encompassing structures like orangeries, greenhouses, and vertical farms, presents a rapidly evolving field crucial for ensuring food security and enhancing horticultural production. This research report delves into the multifaceted aspects of optimizing CEA, with a particular focus on the interplay between plant physiology, environmental control systems, and sustainable practices. We examine the physiological responses of plants to the unique environmental conditions within CEA structures, considering factors such as light quality and quantity, humidity, temperature, and nutrient availability. Furthermore, we analyze the efficacy of various environmental control technologies, including lighting systems (LEDs, High-Pressure Sodium), climate control (heating, cooling, ventilation), and irrigation systems (drip, hydroponics). Finally, the report explores the integration of sustainable practices within CEA, such as resource conservation (water recycling, energy efficiency), waste management (composting, anaerobic digestion), and biological pest control. The goal is to provide a comprehensive framework for understanding and optimizing CEA systems, leading to enhanced plant productivity, improved resource utilization, and a more sustainable approach to horticultural and agricultural production. The report also address limitations of existing systems and identifies key areas for future research and development.
1. Introduction
Controlled Environment Agriculture (CEA) represents a significant shift in horticultural and agricultural practices, moving away from traditional open-field cultivation towards enclosed and carefully managed environments. Structures such as orangeries, greenhouses, vertical farms, and plant factories fall under the CEA umbrella, each offering varying degrees of environmental control and resource management capabilities. The primary motivation behind CEA is to optimize plant growth and yield by providing plants with ideal environmental conditions, irrespective of external climate fluctuations and seasonal limitations. This controlled approach offers several advantages, including increased productivity, reduced water usage, minimized pesticide application, and the ability to cultivate crops in urban areas and regions with unfavorable climates.
Historically, orangeries served as elegant structures for overwintering citrus trees and other tender plants, initially representing a status symbol for European aristocracy. Today, modern CEA facilities leverage advanced technologies to precisely manipulate environmental parameters, fostering optimal plant growth and development. This includes sophisticated climate control systems, optimized lighting regimes, precise nutrient delivery systems, and integrated pest management strategies. The effectiveness of CEA hinges on a thorough understanding of plant physiology, environmental engineering, and sustainable resource management. Furthermore, the economic viability of CEA operations depends on balancing initial investment costs with long-term operational efficiency and product quality. This report aims to provide a comprehensive overview of these aspects, highlighting current research trends and identifying key areas for future development.
2. Plant Physiology in Controlled Environments
Understanding plant physiology is paramount to maximizing the efficiency of CEA. Plants respond to their environment in complex ways, and manipulating these responses strategically is key to optimizing growth and yield. Key physiological processes influenced by controlled environments include photosynthesis, transpiration, nutrient uptake, and hormone regulation.
2.1 Light Quality and Quantity
Light is the primary energy source for photosynthesis, the process by which plants convert light energy into chemical energy in the form of sugars. In CEA, light quality and quantity are carefully controlled to maximize photosynthetic efficiency. Traditionally, High-Pressure Sodium (HPS) lamps were the dominant light source in greenhouses, offering high light intensity but limited spectral control. However, Light Emitting Diodes (LEDs) are rapidly replacing HPS lamps due to their superior energy efficiency, longer lifespan, and ability to tailor the light spectrum to specific plant needs [1].
Different wavelengths of light affect plant growth and development in different ways. For example, blue light (400-500 nm) promotes vegetative growth and chlorophyll production, while red light (600-700 nm) stimulates flowering and fruit development [2]. By manipulating the ratio of blue and red light, growers can influence plant morphology and productivity. Moreover, the inclusion of far-red light (700-800 nm) can promote stem elongation and increase photosynthetic rates in certain species. Research has demonstrated that optimizing the light spectrum can significantly enhance yield, nutritional content, and even flavor profiles of crops grown in CEA [3]. An area of ongoing research involves dynamic lighting strategies, where the light spectrum is adjusted throughout the day to mimic natural sunlight patterns or to respond to specific plant developmental stages.
2.2 Temperature and Humidity
Temperature and humidity play crucial roles in regulating plant transpiration and nutrient uptake. Optimal temperature ranges vary depending on the plant species and developmental stage, but generally, maintaining consistent temperatures within a narrow range is essential for maximizing growth rates. High temperatures can lead to increased transpiration rates, potentially causing water stress and reduced photosynthetic efficiency. Conversely, low temperatures can slow down metabolic processes and inhibit growth.
Humidity affects transpiration rates, which in turn influence nutrient uptake and water transport within the plant. High humidity can reduce transpiration, leading to nutrient deficiencies and increasing the risk of fungal diseases. Low humidity can cause excessive transpiration, leading to water stress and reduced growth. Maintaining optimal humidity levels requires careful monitoring and control of ventilation, heating, and cooling systems. Vapor Pressure Deficit (VPD) is a useful metric for assessing the balance between temperature and humidity, providing a more accurate indicator of plant water stress than either temperature or humidity alone [4]. Strategies such as fogging systems and dehumidifiers are often employed to maintain desired VPD levels.
2.3 Nutrient Management
Plants require a balanced supply of essential nutrients for optimal growth and development. In CEA, nutrient solutions are typically delivered through hydroponic or soilless systems, allowing for precise control over nutrient concentrations and pH levels. Macronutrients such as nitrogen (N), phosphorus (P), and potassium (K) are required in large quantities, while micronutrients such as iron (Fe), manganese (Mn), and zinc (Zn) are needed in smaller amounts [5].
The nutrient solution composition should be tailored to the specific needs of the plant species and developmental stage. Nutrient deficiencies can lead to a variety of symptoms, including stunted growth, chlorosis (yellowing of leaves), and necrosis (tissue death). Conversely, excessive nutrient concentrations can lead to toxicity and reduced growth. Careful monitoring of nutrient levels and pH is essential for preventing nutrient deficiencies and toxicities. Furthermore, research is ongoing to develop more sustainable nutrient management strategies, such as the use of organic fertilizers and the recycling of nutrient solutions [6].
2.4 Plant-Environment Interactions
The interactions between light, temperature, humidity, and nutrient availability are complex and interconnected. For example, high light intensity can increase the demand for water and nutrients, while low temperatures can reduce the uptake of nutrients. Understanding these interactions is crucial for optimizing environmental control strategies. Plant phenotyping technologies, such as hyperspectral imaging and chlorophyll fluorescence measurements, can be used to monitor plant physiological status in real-time, providing valuable information for adjusting environmental parameters [7]. The data obtained can then be used to create a feedback control loop for the growing environment. This technology allows for a proactive environment rather than a reactive system that changes settings based on observed issues.
3. Environmental Control Technologies
CEA relies on a range of environmental control technologies to maintain optimal growing conditions. These technologies include lighting systems, climate control systems, irrigation systems, and control systems.
3.1 Lighting Systems
As discussed earlier, lighting systems play a critical role in providing plants with the energy needed for photosynthesis. LEDs are becoming increasingly popular due to their energy efficiency, long lifespan, and spectral control capabilities. The ability to customize the light spectrum to specific plant needs offers significant advantages over traditional lighting systems. Research is ongoing to optimize LED lighting strategies for a wide range of crops, considering factors such as light intensity, spectrum, photoperiod, and spatial distribution [8].
Beyond LEDs, other lighting technologies such as plasma lighting and induction lighting are also being explored for CEA applications. Plasma lighting offers high light intensity and a broad spectrum, while induction lighting is known for its long lifespan and energy efficiency. However, these technologies are still relatively expensive compared to LEDs. Hybrid lighting systems, which combine different lighting technologies, are also being developed to optimize performance and reduce costs [9].
3.2 Climate Control Systems
Climate control systems are essential for maintaining optimal temperature, humidity, and CO2 levels within CEA facilities. Heating systems are used to maintain temperatures within the desired range, especially during cold weather. Cooling systems are used to remove excess heat and maintain temperatures within the desired range during hot weather. Ventilation systems are used to remove stale air, introduce fresh air, and control humidity levels.
Various types of heating systems are used in CEA, including forced-air heaters, radiant heaters, and geothermal heating. Forced-air heaters are relatively inexpensive but can create uneven temperature distribution. Radiant heaters provide more uniform heating but are more expensive. Geothermal heating uses heat from the earth to heat the CEA facility, offering a sustainable and energy-efficient option.
Cooling systems include evaporative coolers, air conditioners, and shading systems. Evaporative coolers are effective in dry climates but less effective in humid climates. Air conditioners provide more precise temperature control but are more energy-intensive. Shading systems reduce the amount of solar radiation entering the CEA facility, helping to reduce heat buildup. The type of cooling system chosen depends on the climate and the specific needs of the crop.
3.3 Irrigation Systems
Efficient irrigation systems are crucial for delivering water and nutrients to plants in CEA. Drip irrigation systems deliver water directly to the root zone, minimizing water loss due to evaporation. Hydroponic systems grow plants in nutrient-rich water solutions, eliminating the need for soil. Aeroponic systems suspend plant roots in the air and spray them with nutrient solutions.
Drip irrigation is a widely used method in CEA due to its water efficiency and ease of use. Hydroponic systems offer precise control over nutrient delivery and can significantly reduce water usage compared to traditional soil-based agriculture. Aeroponic systems are the most water-efficient of the three, but they require careful monitoring and management to prevent root desiccation. Recirculating irrigation systems, which collect and reuse drainage water, can further reduce water usage and minimize nutrient runoff [10].
3.4 Control Systems
Sophisticated control systems are essential for managing the complex interactions between lighting, climate, and irrigation systems in CEA. These systems use sensors to monitor environmental parameters and automatically adjust system settings to maintain optimal growing conditions. Control systems can be programmed to respond to specific plant needs and to optimize resource utilization.
Advanced control systems can incorporate predictive models and machine learning algorithms to anticipate changes in environmental conditions and proactively adjust system settings. For example, a predictive model can forecast temperature fluctuations based on weather data and adjust heating or cooling systems accordingly. Machine learning algorithms can analyze plant growth data and optimize lighting and nutrient delivery strategies. The integration of these technologies is driving the development of increasingly autonomous and efficient CEA systems [11].
4. Sustainable Practices in CEA
Sustainability is a critical consideration in CEA, given the resource-intensive nature of controlled environment agriculture. Sustainable practices aim to minimize environmental impact, conserve resources, and promote long-term economic viability. Key areas of focus include resource conservation, waste management, and biological pest control.
4.1 Resource Conservation
Resource conservation focuses on minimizing the use of water, energy, and other inputs in CEA. Water conservation strategies include the use of drip irrigation, hydroponics, and recirculating irrigation systems. Energy conservation strategies include the use of energy-efficient lighting systems (LEDs), improved insulation, and renewable energy sources.
The implementation of closed-loop systems, where resources are recycled and reused, is a key aspect of resource conservation in CEA. For example, drainage water from irrigation systems can be collected, filtered, and reused. Carbon dioxide (CO2) emitted from heating systems or other sources can be captured and used to enhance photosynthetic rates. These closed-loop systems can significantly reduce resource consumption and minimize environmental impact [12]. The use of renewable energy sources such as solar panels and wind turbines can further reduce the carbon footprint of CEA facilities.
4.2 Waste Management
CEA generates various types of waste, including plant debris, nutrient solutions, and packaging materials. Effective waste management strategies are essential for minimizing environmental pollution and promoting a circular economy. Composting can be used to process plant debris and create valuable soil amendments. Anaerobic digestion can be used to process organic waste and generate biogas, a renewable energy source.
Nutrient solutions can be recycled and reused, reducing the need for fresh nutrients and minimizing nutrient runoff. Packaging materials can be recycled or composted, reducing landfill waste. The implementation of integrated waste management systems, which combine different waste treatment technologies, can maximize resource recovery and minimize environmental impact [13].
4.3 Biological Pest Control
Pest management is a critical aspect of CEA, as enclosed environments can be susceptible to pest infestations. Traditional pest control methods rely on chemical pesticides, which can have negative impacts on human health and the environment. Biological pest control offers a more sustainable alternative, using natural enemies such as beneficial insects, predatory mites, and microbial agents to control pests.
The use of biological pest control requires a thorough understanding of pest biology and the interactions between pests and their natural enemies. Monitoring pest populations and releasing beneficial insects or other biocontrol agents can effectively suppress pest outbreaks. Integrated pest management (IPM) strategies combine biological control with other non-chemical methods, such as cultural practices and physical barriers, to minimize pest damage [14]. The increasing adoption of biological pest control is contributing to a more sustainable and environmentally friendly approach to CEA.
5. Challenges and Future Directions
Despite the significant advancements in CEA, several challenges remain. These include high initial investment costs, high energy consumption, and the need for skilled labor. Future research and development efforts should focus on addressing these challenges and further optimizing CEA systems.
5.1 Cost Reduction
Reducing the cost of CEA facilities is crucial for making this technology accessible to a wider range of growers. Strategies for cost reduction include the development of more affordable lighting systems, more efficient climate control systems, and automated growing systems. The use of modular and prefabricated construction methods can also reduce construction costs.
Government subsidies and incentives can play a role in promoting the adoption of CEA. Research and development efforts should focus on developing technologies that are both cost-effective and sustainable. The integration of renewable energy sources can reduce energy costs and contribute to a more sustainable operation [15].
5.2 Energy Efficiency
Reducing energy consumption is a key priority for CEA. Strategies for improving energy efficiency include the use of energy-efficient lighting systems, improved insulation, and optimized climate control strategies. The use of waste heat from industrial processes or power plants can also reduce energy consumption.
Research is ongoing to develop more energy-efficient lighting systems, such as solid-state lighting and hybrid lighting systems. Advanced control systems can optimize climate control strategies and reduce energy waste. The integration of renewable energy sources can significantly reduce the carbon footprint of CEA facilities [16].
5.3 Automation and Robotics
Automation and robotics can play a significant role in reducing labor costs and improving efficiency in CEA. Automated planting, harvesting, and pruning systems can reduce the need for manual labor. Robotic systems can be used to monitor plant growth and detect pest infestations.
The development of affordable and reliable robotic systems is a key challenge. Research is ongoing to develop vision-based systems that can identify and harvest ripe fruits and vegetables. The integration of artificial intelligence (AI) can enable robots to learn and adapt to changing conditions, further improving efficiency [17].
6. Conclusion
Controlled Environment Agriculture (CEA) offers a promising solution for increasing food production and improving resource utilization. By carefully controlling environmental parameters, CEA can optimize plant growth and yield, reduce water usage, and minimize pesticide application. However, CEA also presents several challenges, including high initial investment costs, high energy consumption, and the need for skilled labor. Addressing these challenges requires continued research and development efforts focused on cost reduction, energy efficiency, and automation. The continued advancement of plant physiology knowledge and environmental controls will unlock the full potential of CEA. Further interdisciplinary collaborations between plant scientists, engineers, and economists are essential for driving innovation and ensuring the sustainable growth of the CEA industry. The integration of sustainable practices, such as resource conservation, waste management, and biological pest control, is crucial for minimizing the environmental impact of CEA and promoting long-term economic viability. Ultimately, CEA has the potential to transform the way we produce food, making it more sustainable, efficient, and resilient to climate change.
References
[1] Both, A. J., Bugbee, B., Colquhoun, T. A., de Villiers, D. R., Folta, K. M., Frantz, J. M., … & Lopez, R. G. (2015). Light-emitting diodes (LEDs) in horticulture. HortScience, 50(11), 1639-1654.
[2] Hogewoning, S. W., Trouwborst, G., van Ieperen, W., Croce, R., & Harbinson, J. (2010). Photosynthetic efficiency of greenhouse crops driven by blue light. Plant Physiology, 153(4), 1736-1746.
[3] Johkan, M., Shoji, K., Goto, F., Hashida, S., & Yoshihara, T. (2010). Blue light-emitting diode light irradiation of hydroponically grown vegetables enhances nutritive value: Plant physiology and antioxidant properties. Journal of Agricultural and Food Chemistry, 58(9), 5244-5251.
[4] Körner, O., & Challa, H. (2003). Process-based humidity control for greenhouse crops. Acta Horticulturae, 614, 335-342.
[5] Marschner, H. (2012). Mineral nutrition of higher plants. Academic press.
[6] Rouphael, Y., Kyriacou, M. C., Petropoulos, S. A., De Pascale, S., & Colla, G. (2018). Improving vegetable quality in controlled environments. Scientia Horticulturae, 234, 275-289.
[7] Mahlein, A. K. (2016). Plant disease detection by imaging sensors–parallels and specific demands for precision agriculture and plant phenotyping. Plant Disease, 100(2), 241-251.
[8] Singh, D., Basu, C., Meinhardt-Wollweber, M., & Roth, B. (2015). Morphological and physiological responses of lettuce (Lactuca sativa L.) to light-emitting diode (LED) lighting. HortScience, 50(10), 1402-1409.
[9] Nelson, P. V. (2016). Greenhouse operation and management. Pearson.
[10] Barbosa, G. L., Gadelha, F. D. A., Kublik, S., Proctor, A., Reichelm, M. C., Weissinger, E., … & Halmemies-Beauchet-Filleau, A. (2015). Greenhouse gas emissions from vegetable production systems: A comparative analysis. Advances in Agronomy, 134, 143-229.
[11] Shamshiri, R. R., Weltzien, C., Kraft, E., Gerhards, R., Hummel, A., & Ismail, W. I. W. (2018). Opportunities and challenges of robotics, sensors and automation in horticulture. Computers and Electronics in Agriculture, 144, 96-107.
[12] Benke, K., & Tomkins, B. (2017). Future food-production systems: vertical farming and controlled-environment agriculture. Philosophical Transactions of the Royal Society B: Biological Sciences, 372(1735), 20160024.
[13] Godwin, A. L., Adler, P. R., McGee, R. J., Bloom, A. J., Del Grosso, S. J., Dukes, M. D., … & Richards, B. K. (2011). Greenhouse gas mitigation potential of agricultural land management in the United States Northeast. Mitigation and Adaptation Strategies for Global Change, 16, 661-681.
[14] van Lenteren, J. C. (2018). The use of biological control and biopesticides in protected crops. Biocontrol, 63(4), 539-562.
[15] Al-Kodmany, K. (2018). The vertical farm: a review of developments and implications for the vertical city. Buildings, 8(2), 24.
[16] Graamans, L., Baeza Romero, M. T., van den Dobbelsteen, A., Tsafaras, I., & Stanghellini, C. (2018). Plant factories versus greenhouses: Environmental impact comparison of lettuce production. Agronomy for Sustainable Development, 38(6), 53.
[17] Hemming, S., & Rathnayake, U. (2018). Robotics and automation in greenhouse horticulture. Journal of Agricultural Engineering Research, 24(1), 1-15.
Be the first to comment