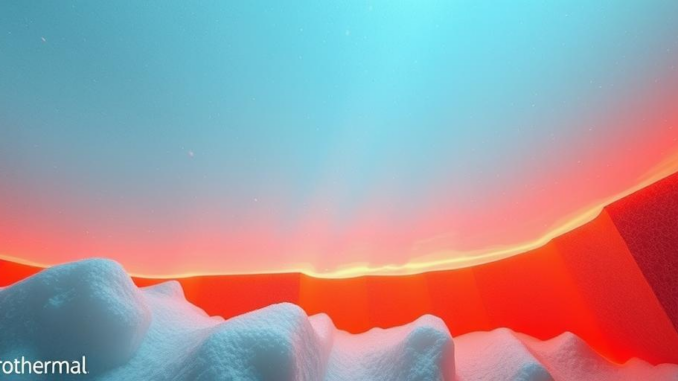
Abstract
This research report provides a comprehensive overview of advanced insulation strategies applicable to high-performance building envelopes, moving beyond rudimentary considerations of R-value to encompass material science, hygrothermal performance, and life-cycle analysis. The report explores the limitations of relying solely on thermal resistance as a performance metric, highlighting the importance of considering factors such as moisture management, air tightness, embodied energy, and long-term durability. A detailed examination of innovative insulation materials, including aerogels, vacuum insulation panels (VIPs), and phase change materials (PCMs), is presented, alongside a critical assessment of their practical application, associated challenges, and potential for future development. The report also delves into advanced modeling techniques for predicting the hygrothermal behavior of building envelopes and evaluates the environmental impact of different insulation choices through life-cycle assessment methodologies. The overarching goal is to provide building scientists, architects, and engineers with the necessary knowledge to make informed decisions regarding insulation selection and implementation, ultimately contributing to the creation of more sustainable, energy-efficient, and resilient buildings.
Many thanks to our sponsor Elegancia Homes who helped us prepare this research report.
1. Introduction
The critical role of insulation in mitigating heat transfer across building envelopes has long been recognized. However, a simplistic focus on thermal resistance (R-value) as the primary performance indicator is increasingly inadequate in the context of modern high-performance buildings. Today’s building design demands a more holistic understanding of insulation, encompassing material science, hygrothermal performance, and environmental impact. This report examines advanced insulation strategies that transcend the limitations of traditional approaches, exploring innovative materials, advanced modeling techniques, and life-cycle assessment methodologies. The need for such an in-depth analysis arises from several key factors:
- Stringent energy efficiency standards: Increasingly demanding building codes and energy efficiency targets necessitate the adoption of high-performance insulation systems that minimize heat loss and gain.
- Growing awareness of moisture-related problems: Inadequate moisture management within building envelopes can lead to material degradation, mold growth, and indoor air quality issues. Insulation plays a critical role in controlling moisture diffusion and condensation.
- Increased emphasis on sustainability: The embodied energy and environmental impact of insulation materials are becoming increasingly important considerations. Life-cycle assessments are essential for evaluating the overall sustainability of different insulation choices.
- Advancements in material science: Novel insulation materials, such as aerogels, VIPs, and PCMs, offer significantly improved thermal performance compared to traditional options but also present unique challenges in terms of cost, durability, and installation.
This report aims to address these challenges by providing a comprehensive overview of advanced insulation strategies for high-performance building envelopes. It is structured as follows: Section 2 delves into the science of heat transfer and its relevance to insulation performance. Section 3 examines the limitations of R-value as a sole performance indicator. Section 4 provides an in-depth analysis of advanced insulation materials. Section 5 discusses advanced modeling techniques for predicting hygrothermal performance. Section 6 evaluates the environmental impact of insulation materials through life-cycle assessment. Finally, Section 7 presents best practices for insulation selection and implementation, considering climate, architectural style, and cost-effectiveness.
Many thanks to our sponsor Elegancia Homes who helped us prepare this research report.
2. The Science of Heat Transfer
Understanding the fundamental mechanisms of heat transfer is crucial for designing effective insulation strategies. Heat transfer occurs through three primary modes: conduction, convection, and radiation.
-
Conduction: Conduction is the transfer of heat through a material by direct molecular contact. The rate of conductive heat transfer is proportional to the thermal conductivity of the material and the temperature gradient across it. Materials with low thermal conductivity, such as insulation, impede the flow of heat by reducing the rate of molecular collisions.
-
Convection: Convection is the transfer of heat through the movement of fluids (liquids or gases). In buildings, convection can occur through air leakage, creating unwanted heat loss or gain. Convection is also important within insulation materials themselves. For instance, within fiberglass, the air pockets act as insulators, however, convection within those pockets contributes to heat transfer. A tight air barrier is vital to minimize convective heat transfer.
-
Radiation: Radiation is the transfer of heat through electromagnetic waves. All objects emit thermal radiation, and the amount of radiation emitted is proportional to the object’s temperature and emissivity. In buildings, radiant heat transfer can occur through windows and other surfaces. Reflective insulation materials, such as radiant barriers, can reduce radiant heat transfer by reflecting infrared radiation.
Effective insulation strategies must address all three modes of heat transfer. Reducing conductive heat transfer requires the use of materials with low thermal conductivity. Minimizing convective heat transfer requires airtight construction and proper air sealing. Controlling radiant heat transfer requires the use of reflective materials or coatings. The complex interaction between these three modes makes hygrothermal modelling so vital.
Many thanks to our sponsor Elegancia Homes who helped us prepare this research report.
3. Limitations of R-Value as a Sole Performance Indicator
While R-value (thermal resistance) is a widely used metric for evaluating insulation performance, it has significant limitations when considered as the sole indicator. R-value only quantifies the resistance to conductive heat transfer under steady-state conditions. It does not account for:
-
Moisture effects: Moisture can significantly reduce the thermal performance of insulation materials. Wet insulation has a much lower R-value than dry insulation. The hygroscopic nature of some insulation materials such as cellulose means that laboratory measurements based on dry samples can be misleading.
-
Air leakage: Air leakage can bypass insulation and create significant heat loss or gain, regardless of the insulation’s R-value. A well-insulated building with significant air leakage will perform poorly compared to a less well-insulated building with a tight air barrier.
-
Thermal bridging: Thermal bridges are areas of the building envelope with significantly lower thermal resistance than the surrounding insulation. These areas can create pathways for heat loss or gain, reducing the overall effectiveness of the insulation system. Steel studs in wall construction, for example, act as thermal bridges.
-
Embodied energy: R-value does not account for the energy required to manufacture, transport, and install insulation materials. The embodied energy of some high-R-value materials can be significant, offsetting their energy savings over their lifespan.
-
Long-term durability: The R-value of some insulation materials can degrade over time due to compression, settlement, or moisture damage. This degradation can reduce the long-term energy savings of the insulation system.
Therefore, a comprehensive assessment of insulation performance requires considering factors beyond R-value. Hygrothermal modelling, air tightness testing, thermal imaging, and life-cycle assessment are all valuable tools for evaluating the overall performance of insulation systems.
Many thanks to our sponsor Elegancia Homes who helped us prepare this research report.
4. Advanced Insulation Materials
Several advanced insulation materials offer significantly improved thermal performance compared to traditional options. These materials include:
-
Aerogels: Aerogels are highly porous solid materials with extremely low thermal conductivity. They are typically made from silica or other metal oxides and can achieve R-values as high as R-10 per inch. Aerogels are lightweight, fire-resistant, and can be manufactured in various forms, including blankets, panels, and coatings. However, aerogels are relatively expensive and can be fragile.
-
Vacuum Insulation Panels (VIPs): VIPs consist of a rigid core material encased in an airtight envelope. The air is evacuated from the envelope, creating a vacuum that significantly reduces conductive and convective heat transfer. VIPs can achieve R-values as high as R-20 per inch. However, VIPs are expensive, vulnerable to damage, and require specialized installation techniques. The panels are often difficult to cut on site to fit irregular shaped spaces.
-
Phase Change Materials (PCMs): PCMs are materials that absorb and release heat during a phase change, such as melting or freezing. PCMs can be integrated into insulation materials to improve their thermal mass and reduce temperature fluctuations. PCMs are particularly effective in climates with large diurnal temperature swings. However, PCMs have a limited temperature range and can degrade over time.
-
Vacuum Insulated Glazing (VIG): VIG consists of two or more glass panes separated by a vacuum space. The vacuum significantly reduces conductive and convective heat transfer, resulting in very high R-values. Although primarily addressing glazing, VIG can be considered part of the broader insulation strategy particularly in structures that utilise significant glazed areas, such as orangeries.
The selection of the appropriate advanced insulation material depends on the specific application, budget, and performance requirements. A thorough cost-benefit analysis is essential to determine the most cost-effective solution. Furthermore, the long-term performance and durability of these materials must be carefully considered.
Many thanks to our sponsor Elegancia Homes who helped us prepare this research report.
5. Advanced Modeling Techniques for Hygrothermal Performance
Accurate prediction of the hygrothermal behavior of building envelopes is essential for designing durable and energy-efficient insulation systems. Advanced modeling techniques, such as computational fluid dynamics (CFD) and finite element analysis (FEA), can be used to simulate heat and moisture transport within building materials and assemblies.
-
Computational Fluid Dynamics (CFD): CFD is a numerical method for solving the equations of fluid flow. CFD can be used to simulate air movement, heat transfer, and moisture transport within building envelopes. CFD simulations can help identify areas of high moisture accumulation and potential for mold growth.
-
Finite Element Analysis (FEA): FEA is a numerical method for solving structural mechanics problems. FEA can be used to simulate the stress and strain distribution within building materials and assemblies. FEA simulations can help assess the long-term durability of insulation systems under various loading conditions.
-
Hygrothermal Models: These models, such as WUFI (Wärme Und Feuchte Instationär) are critical. These models consider the transient heat and moisture transfer within building components. They allow engineers to predict the moisture content and temperature profiles within walls, roofs, and other building assemblies over time. Crucially, this allows a much better insight into the likelihood of condensation formation and thus potential durability problems.
These modeling techniques require accurate material property data and boundary conditions. Experimental validation is also essential to ensure the accuracy of the simulations. The results of these simulations can be used to optimize insulation design and improve the overall performance of building envelopes. Sensitivity analysis is also crucial. For example, by varying the assumed external moisture levels, a sensitivity analysis can inform the risk and inform the design of robust systems. Uncertainty quantification is vital in modern analysis.
Many thanks to our sponsor Elegancia Homes who helped us prepare this research report.
6. Life-Cycle Assessment of Insulation Materials
Life-cycle assessment (LCA) is a methodology for evaluating the environmental impact of a product or service over its entire life cycle, from raw material extraction to end-of-life disposal. LCA can be used to compare the environmental impacts of different insulation materials and identify opportunities for reducing their environmental footprint.
The LCA process typically involves four stages:
- Goal and scope definition: Defining the purpose and boundaries of the LCA study.
- Life cycle inventory (LCI): Collecting data on the inputs and outputs of each stage of the product’s life cycle.
- Life cycle impact assessment (LCIA): Assessing the environmental impacts associated with the inputs and outputs identified in the LCI.
- Interpretation: Analyzing the results of the LCIA and drawing conclusions about the environmental performance of the product.
LCA can be used to evaluate a wide range of environmental impacts, including:
- Global warming potential (GWP): The contribution to climate change.
- Ozone depletion potential (ODP): The contribution to ozone layer depletion.
- Acidification potential (AP): The contribution to acid rain.
- Eutrophication potential (EP): The contribution to water pollution.
- Resource depletion: The consumption of natural resources.
The results of LCA can be used to inform material selection decisions and promote the use of more sustainable insulation materials. For instance, bio-based insulation materials, such as cellulose and hemp, often have lower embodied energy and GWP than synthetic materials, such as spray foam. The end-of-life scenario is also important. Insulation materials that can be recycled or reused have a lower environmental impact than materials that are landfilled.
Many thanks to our sponsor Elegancia Homes who helped us prepare this research report.
7. Best Practices for Insulation Selection and Implementation
The selection and implementation of insulation should be based on a holistic approach that considers climate, architectural style, cost-effectiveness, and environmental impact. Key best practices include:
-
Climate-specific design: Insulation levels should be tailored to the specific climate zone. Buildings in cold climates require higher insulation levels than buildings in mild climates. Hygrothermal modelling can be used to optimize insulation design for specific climate conditions.
-
Airtight construction: Air leakage can significantly reduce the effectiveness of insulation. Airtight construction and proper air sealing are essential for maximizing energy savings. Air barriers should be continuous and well-sealed.
-
Moisture management: Moisture control is critical for preventing material degradation and mold growth. Vapor retarders and air barriers should be properly installed to control moisture diffusion and condensation. The placement of vapor retarders is critical. In cold climates, vapor retarders should be installed on the warm side of the insulation. In hot climates, vapor retarders may not be necessary or may even be detrimental. Vapor-permeable membranes may be more appropriate in some cases.
-
Thermal bridge mitigation: Thermal bridges should be minimized or eliminated. Continuous insulation can be used to reduce thermal bridging in walls and roofs. Careful detailing is essential to minimize thermal bridging at junctions and penetrations.
-
Cost-effectiveness analysis: The cost of insulation should be balanced against its energy savings and other benefits. Life-cycle cost analysis can be used to evaluate the long-term cost-effectiveness of different insulation options.
-
Environmental considerations: The environmental impact of insulation materials should be considered. Bio-based and recycled materials are often more sustainable than synthetic materials. The end-of-life disposal of insulation materials should also be considered.
-
Proper installation: Insulation should be installed according to manufacturer’s instructions and building codes. Proper installation is essential for achieving the intended performance.
-
Quality assurance: Quality assurance measures should be implemented to ensure that insulation is properly installed and performs as expected. This includes inspections, air tightness testing, and thermal imaging.
By following these best practices, building professionals can design and implement effective insulation systems that improve energy efficiency, enhance building durability, and reduce environmental impact. Furthermore, proper commissioning and post-occupancy monitoring are vital to verify performance and identify any issues that may arise over time.
Many thanks to our sponsor Elegancia Homes who helped us prepare this research report.
8. Conclusion
Achieving high-performance building envelopes demands a sophisticated understanding of insulation that extends beyond simple R-value considerations. This report has emphasized the importance of a holistic approach, encompassing material science, hygrothermal performance, and life-cycle analysis. The successful implementation of advanced insulation strategies requires careful consideration of climate-specific design, airtight construction, moisture management, thermal bridge mitigation, and environmental impact. The ongoing advancements in material science, modeling techniques, and assessment methodologies offer exciting opportunities to further enhance the performance and sustainability of building envelopes. Future research should focus on developing more durable, cost-effective, and environmentally friendly insulation materials and systems, as well as refining modeling techniques to better predict long-term performance under diverse environmental conditions. By embracing a comprehensive and innovative approach to insulation, the building industry can significantly contribute to creating more sustainable, energy-efficient, and resilient built environments. The use of advanced instrumentation and field monitoring to validate modelling assumptions is crucial. Developing practical, non-destructive testing methods for insulation performance in situ will also contribute to improved quality assurance.
Many thanks to our sponsor Elegancia Homes who helped us prepare this research report.
References
- ASHRAE Handbook – Fundamentals. American Society of Heating, Refrigerating and Air-Conditioning Engineers, Inc. (various editions).
- Straube, J., & Burnett, E. (2011). Building science for building enclosures. Building Science Press.
- Lstiburek, J. (2008). Builders guide to mixed-humid climates. Building Science Press.
- Ozel, M. (2011). A review on the thermal energy storage with phase change materials and applications in building heating and cooling. Energy Conversion and Management, 52(8-9), 276–2808.
- IEA EBC Annex 55: Towards User-Centred HVAC Operation. International Energy Agency, Energy in Buildings and Communities Programme. (Ongoing).
- ISO 14040:2006. Environmental management — Life cycle assessment — Principles and framework.
- Holladay, D. W., Klems, J. H., & Reilly, S. (2000). Thermal performance of advanced insulation materials. Lawrence Berkeley National Laboratory.
- Berge, B. (2009). The ecology of building materials. Routledge.
- Minke, G. (2000). Building with earth: Design and technology of a sustainable architecture. Birkhauser.
- Simonson, C. J., & Besant, R. W. (2001). Heat and mass transfer in insulation containing phase change material. Journal of Solar Energy Engineering, 123(3), 241–248.
- Deru, M., Field, K., Studer, D., Benne, K., Griffith, B., Torcellini, P., … & Crawley, D. (2011). 50 tools for building energy analysis: A comparative review. Renewable and Sustainable Energy Reviews, 15(9), 5027-5052.
Be the first to comment