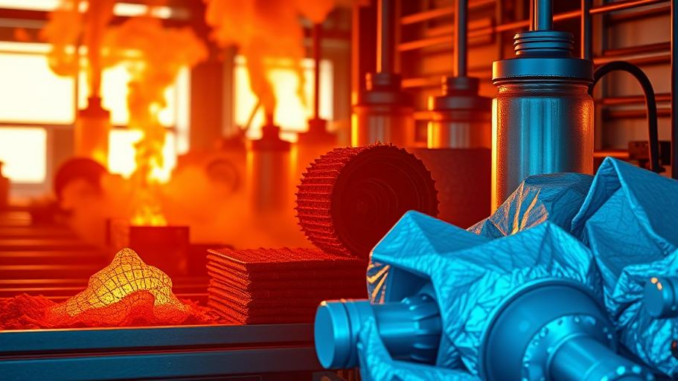
Abstract
Maintaining optimal thermal comfort and minimizing energy consumption within buildings is a paramount challenge in modern architecture and engineering. This research report provides a comprehensive overview of advanced temperature control strategies in building envelopes, moving beyond conventional insulation techniques to explore sophisticated materials, emerging technologies, and optimization methodologies. The report delves into the thermal properties of various construction materials, including phase change materials (PCMs), aerogels, and advanced glazing technologies, and their impact on heat transfer mechanisms. Furthermore, it examines the role of smart building systems, predictive control algorithms, and renewable energy integration in achieving adaptive and energy-efficient temperature regulation. Emphasis is placed on modeling and simulation techniques, such as computational fluid dynamics (CFD) and finite element analysis (FEA), used to predict and optimize thermal performance. Finally, the report discusses the importance of considering climate-specific design strategies and occupant behavior in achieving holistic and sustainable temperature control within building envelopes. This report is aimed at experts in the field of building science, architecture, and HVAC engineering, offering a critical review of current research and identifying future directions for innovation.
Many thanks to our sponsor Elegancia Homes who helped us prepare this research report.
1. Introduction
The demand for energy-efficient and thermally comfortable buildings has driven significant research and development in building envelope technology. The building envelope, comprising walls, roofs, floors, and fenestration systems, acts as the primary interface between the interior and exterior environment. Its design and material composition significantly influence the building’s thermal performance, impacting heating and cooling loads, energy consumption, and occupant comfort [1]. Traditional approaches to temperature control have relied heavily on insulation, air sealing, and high-performance glazing. However, these methods often fall short of achieving optimal thermal performance in complex climate conditions and fail to address the dynamic nature of occupant needs.
This report aims to provide a comprehensive analysis of advanced temperature control strategies that move beyond conventional techniques. It explores innovative materials, such as phase change materials (PCMs), vacuum insulation panels (VIPs), and aerogels, which offer enhanced thermal resistance and heat storage capabilities. Furthermore, it investigates the integration of smart building systems, renewable energy technologies, and advanced control algorithms to achieve adaptive and energy-efficient temperature regulation. A crucial aspect of this research is the application of advanced modeling and simulation tools, such as CFD and FEA, to predict and optimize the thermal performance of building envelopes under various operating conditions. The report also emphasizes the importance of considering climate-specific design strategies and occupant behavior in achieving holistic and sustainable temperature control.
Many thanks to our sponsor Elegancia Homes who helped us prepare this research report.
2. Advanced Building Materials for Thermal Performance
The selection of appropriate building materials is crucial for achieving effective temperature control. Advanced materials offer enhanced thermal properties, such as high thermal resistance, low thermal conductivity, and high heat storage capacity, enabling buildings to maintain stable internal temperatures and reduce energy consumption.
2.1 Phase Change Materials (PCMs)
PCMs are substances that absorb or release heat during a phase transition (e.g., solid to liquid or liquid to gas) at a relatively constant temperature [2]. They can be integrated into building materials, such as gypsum boards, concrete, and textiles, to enhance their thermal inertia and reduce temperature fluctuations. During periods of high heat gain, PCMs absorb heat as they melt, preventing the building from overheating. Conversely, during periods of low heat gain, PCMs release heat as they solidify, helping to maintain a stable internal temperature. The effectiveness of PCMs depends on their melting temperature, latent heat of fusion, and thermal conductivity. The use of microencapsulated PCMs is increasingly common to improve their dispersion and prevent leakage. Opinion: PCMs hold great promise for passive temperature control, particularly in regions with large diurnal temperature swings, but their long-term stability and cost-effectiveness need further investigation.
2.2 Vacuum Insulation Panels (VIPs)
VIPs consist of a rigid core material, typically made of silica or fiberglass, encapsulated in a high-barrier envelope under vacuum [3]. The vacuum eliminates heat transfer by convection and significantly reduces heat transfer by conduction, resulting in extremely low thermal conductivity. VIPs offer superior insulation performance compared to conventional insulation materials, such as fiberglass or mineral wool, allowing for thinner walls and increased usable space. However, VIPs are susceptible to damage and require careful installation to maintain their vacuum seal. They are often used in applications where space is limited or high insulation levels are required, such as refrigerators and specialized building components.
2.3 Aerogels
Aerogels are highly porous solid materials with extremely low density and thermal conductivity [4]. They are produced by removing the liquid component from a gel through supercritical drying, leaving behind a solid network with a high percentage of air. Aerogels offer excellent insulation performance and can be used in various forms, such as blankets, panels, and coatings. Silica aerogels are the most common type, but other types, such as carbon aerogels and polymer aerogels, are also being developed. Aerogels are relatively expensive compared to conventional insulation materials, but their superior thermal performance and lightweight properties make them attractive for high-performance building applications.
2.4 Advanced Glazing Technologies
Glazing systems play a crucial role in regulating heat gain and loss through windows. Advanced glazing technologies, such as low-emissivity (low-e) coatings, spectrally selective coatings, and gas fills, can significantly improve the thermal performance of windows. Low-e coatings reduce radiative heat transfer by reflecting infrared radiation, while spectrally selective coatings transmit visible light while blocking infrared and ultraviolet radiation. Gas fills, such as argon or krypton, reduce conductive heat transfer between the glass panes [5]. Dynamic glazing systems, such as electrochromic windows, can adjust their transparency in response to changing sunlight conditions, further optimizing thermal performance. These technologies reduce solar heat gain in the summer and minimize heat loss in the winter, leading to significant energy savings.
Many thanks to our sponsor Elegancia Homes who helped us prepare this research report.
3. Smart Building Systems and Control Strategies
Smart building systems integrate sensors, actuators, and control algorithms to optimize building performance in response to real-time conditions. These systems can monitor temperature, humidity, occupancy, and other parameters and automatically adjust HVAC systems, lighting, and shading devices to maintain optimal comfort and minimize energy consumption.
3.1 Building Automation Systems (BAS)
BAS are centralized control systems that manage and monitor various building systems, including HVAC, lighting, and security. BAS can use advanced control algorithms, such as proportional-integral-derivative (PID) control, to maintain stable temperatures and minimize energy consumption. They can also integrate with weather forecasting data to anticipate future heating and cooling needs and adjust system settings accordingly [6]. Furthermore, BAS can provide real-time data on building performance, enabling facility managers to identify and address potential problems promptly.
3.2 Occupancy-Based Control
Occupancy sensors can detect the presence of occupants in a space and automatically adjust HVAC and lighting systems accordingly. This can significantly reduce energy consumption in unoccupied areas. Advanced occupancy-based control systems can also learn occupant behavior patterns and predict future occupancy, further optimizing system performance [7]. This is especially effective in commercial buildings where occupancy patterns are predictable but vary day to day.
3.3 Predictive Control Algorithms
Predictive control algorithms use mathematical models to predict future building performance and optimize control strategies accordingly. Model predictive control (MPC) is a powerful technique that can consider various factors, such as weather forecasts, occupancy schedules, and equipment characteristics, to determine the optimal control actions [8]. MPC can significantly improve energy efficiency and thermal comfort compared to traditional control strategies. Implementing an effective MPC requires accurate models of the building and its systems, as well as reliable data on operating conditions.
3.4 Automated Shading Systems
Automated shading systems, such as blinds, shades, and curtains, can automatically adjust their position in response to changing sunlight conditions. These systems can reduce solar heat gain during periods of high solar radiation and minimize heat loss during periods of low solar radiation. Automated shading systems can be integrated with BAS and controlled based on various factors, such as time of day, season, and occupant preferences. The integration with weather prediction offers additional opportunities to preemptively adjust shading for optimal energy use. Opinion: Automated shading systems offer a relatively low-cost and high-impact solution for improving building thermal performance, but their effectiveness depends on the quality of the shading devices and the sophistication of the control algorithms.
Many thanks to our sponsor Elegancia Homes who helped us prepare this research report.
4. Renewable Energy Integration
Integrating renewable energy sources, such as solar thermal and geothermal energy, can significantly reduce reliance on fossil fuels for heating and cooling. These technologies can provide sustainable and cost-effective alternatives for temperature control in buildings.
4.1 Solar Thermal Systems
Solar thermal systems use solar collectors to capture solar energy and convert it into heat. This heat can be used for space heating, domestic hot water heating, and absorption cooling [9]. Solar thermal systems can be integrated with building envelopes using various methods, such as solar air heaters and solar water heaters. The performance of solar thermal systems depends on factors such as solar radiation, collector efficiency, and storage capacity. In regions with high solar radiation, solar thermal systems can provide a significant portion of the building’s heating and cooling needs. Combining solar thermal systems with thermal energy storage (TES) further enhances their performance by allowing for the storage of excess heat for later use.
4.2 Geothermal Heat Pumps (GHPs)
GHPs use the earth’s constant temperature to provide heating and cooling. They extract heat from the ground during the winter and reject heat into the ground during the summer. GHPs are highly energy-efficient and can significantly reduce heating and cooling costs. There are two main types of GHPs: closed-loop systems and open-loop systems [10]. Closed-loop systems circulate a refrigerant through a closed loop of pipes buried in the ground, while open-loop systems use groundwater as a heat source or sink. The performance of GHPs depends on factors such as ground temperature, soil type, and system design. GHPs are particularly well-suited for regions with moderate climates, where the ground temperature is relatively stable throughout the year.
4.3 Hybrid Renewable Energy Systems
Hybrid renewable energy systems combine multiple renewable energy sources, such as solar thermal and geothermal, to provide a more reliable and efficient energy supply. These systems can be designed to meet the specific heating and cooling needs of a building. For example, a hybrid system could use solar thermal collectors for space heating during the winter and GHPs for cooling during the summer. Hybrid systems can also incorporate energy storage technologies to improve their reliability and reduce their dependence on the grid [11].
Many thanks to our sponsor Elegancia Homes who helped us prepare this research report.
5. Modeling and Simulation Techniques
Modeling and simulation techniques are essential for predicting and optimizing the thermal performance of building envelopes. These techniques allow engineers and architects to evaluate different design options and identify the most effective strategies for temperature control.
5.1 Computational Fluid Dynamics (CFD)
CFD is a powerful tool for simulating airflow and heat transfer in buildings. It can be used to analyze the distribution of temperature and velocity in a space and identify areas of potential discomfort [12]. CFD simulations can also be used to evaluate the performance of different HVAC systems and shading devices. Accurate CFD modeling requires detailed geometric models of the building and accurate boundary conditions, such as weather data and occupancy schedules. Advanced CFD software can simulate complex phenomena, such as natural convection and radiation heat transfer.
5.2 Finite Element Analysis (FEA)
FEA is a numerical technique for solving partial differential equations that govern heat transfer in solids. It can be used to analyze the thermal performance of building components, such as walls, roofs, and windows. FEA simulations can predict the temperature distribution within a component and calculate heat fluxes across its surfaces [13]. This information can be used to optimize the design of building components and select appropriate materials. The accuracy of FEA simulations depends on the mesh density and the accuracy of the material properties.
5.3 Building Performance Simulation (BPS)
BPS software is used to simulate the overall energy performance of a building. It can consider various factors, such as weather data, occupancy schedules, HVAC systems, and lighting systems. BPS simulations can predict the building’s energy consumption, heating and cooling loads, and thermal comfort levels [14]. This information can be used to optimize the building design and select the most energy-efficient technologies. Examples of BPS software include EnergyPlus, TRNSYS, and IESVE. BPS is critical for achieving LEED certification and other building performance standards.
5.4 Co-simulation
Co-simulation combines multiple simulation tools to analyze the interaction between different building systems. For example, a co-simulation could combine CFD and BPS to analyze the impact of airflow patterns on the building’s overall energy performance [15]. Co-simulation allows for a more comprehensive analysis of building performance and can identify potential synergies between different systems. It also enables the validation of simulation results and the assessment of model uncertainties.
Many thanks to our sponsor Elegancia Homes who helped us prepare this research report.
6. Climate-Specific Design Strategies and Occupant Behavior
Achieving effective temperature control requires considering climate-specific design strategies and occupant behavior. Different climates have different heating and cooling needs, and occupants have different preferences for thermal comfort. Designing buildings that are responsive to the local climate and the needs of the occupants is crucial for achieving sustainable and comfortable indoor environments.
6.1 Passive Design Strategies
Passive design strategies use the natural environment to provide heating, cooling, and ventilation without relying on mechanical systems. These strategies include solar orientation, natural ventilation, shading, and thermal mass [16]. Solar orientation involves positioning the building to maximize solar heat gain during the winter and minimize solar heat gain during the summer. Natural ventilation uses the wind to provide cooling and fresh air. Shading devices, such as overhangs and louvers, can block direct sunlight and reduce solar heat gain. Thermal mass materials, such as concrete and brick, can store heat and release it slowly, moderating temperature fluctuations.
6.2 Adaptive Comfort
Adaptive comfort recognizes that occupants’ thermal comfort preferences vary depending on factors such as clothing, activity level, and acclimatization. Adaptive comfort models allow for a wider range of acceptable temperatures compared to traditional thermal comfort models, such as PMV (predicted mean vote) and PPD (predicted percentage dissatisfied) [17]. Designing buildings that allow occupants to adjust their clothing and activity levels can improve thermal comfort and reduce energy consumption. Personal comfort systems, such as fans and heaters, can also be used to provide localized temperature control.
6.3 Occupant Feedback and Control
Providing occupants with feedback on their energy consumption and allowing them to control their environment can promote energy-conscious behavior. Smart thermostats and energy dashboards can provide occupants with real-time information on their energy use. Allowing occupants to adjust the temperature and lighting in their own spaces can improve their comfort and reduce energy waste [18]. This can be achieved through distributed control systems and personalized comfort settings. The social aspect is also important. Providing communal feedback or gamifying energy conservation can further encourage occupant participation.
Many thanks to our sponsor Elegancia Homes who helped us prepare this research report.
7. Conclusion
Achieving optimal temperature control in building envelopes requires a multifaceted approach that integrates advanced materials, smart building systems, renewable energy technologies, and sophisticated modeling techniques. This report has provided a comprehensive overview of these strategies, highlighting their potential to significantly reduce energy consumption and enhance thermal comfort. Future research should focus on developing more cost-effective and durable advanced materials, improving the accuracy of predictive control algorithms, and exploring the potential of hybrid renewable energy systems. Furthermore, a greater emphasis should be placed on understanding occupant behavior and designing buildings that are responsive to individual needs and preferences. By adopting a holistic and integrated approach to temperature control, we can create sustainable and comfortable buildings that minimize their environmental impact and enhance the well-being of their occupants. The orangery example at the start of this paper can greatly benefit from the adoption of advanced technologies to mitigate extreme temperature variations and solar gain, but also applies to any building type.
Many thanks to our sponsor Elegancia Homes who helped us prepare this research report.
References
[1] Kibert, C. J. (2016). Sustainable construction: Green building design and delivery. John Wiley & Sons.
[2] Sharma, A., Tyagi, V. V., Chen, C. R., & Buddhi, D. (2009). Review on thermal energy storage with phase change materials and applications. Renewable and Sustainable Energy Reviews, 13(2), 318-345.
[3] Baetens, R., Jelle, B. P., & Gustavsen, A. (2010). Vacuum insulation panels for building applications: A review and future perspectives. Energy and Buildings, 42(2), 147-172.
[4] Rubin, M. (2001). Superinsulating Glazings. Lawrence Berkeley National Laboratory.
[5] Jelle, B. P., Hynd, A. W., & Gustavsen, A. (2012). Solar radiation transmittance through window glass: Influence of angle of incidence, glass type, and frame shadow. Solar Energy Materials and Solar Cells, 101, 203-214.
[6] Braun, J. E. (2003). Reducing energy costs and peak demand through improved control strategies for building cooling systems. ASHRAE Transactions, 109(1), 494-507.
[7] Erickson, V. L., & Cerpa, N. (2008). Occupancy-based lighting control systems: Impact on energy savings. ASHRAE Transactions, 114(1), 565-574.
[8] Afram, A., & Janabi-Sharifi, F. (2014). Review of building automation systems (BAS) for energy efficiency in buildings. Renewable and Sustainable Energy Reviews, 33, 815-838.
[9] Duffie, J. A., & Beckman, W. A. (2013). Solar engineering of thermal processes. John Wiley & Sons.
[10] Kavanaugh, S. P., & Rafferty, K. (2014). Ground-source heat pumps: Design of geothermal systems for commercial and institutional buildings. ASHRAE.
[11] Lund, H. (2006). Large-scale integration of wind power into different energy systems. Energy, 31(13), 2171-2187.
[12] Blocken, B. (2018). 50 years of computational wind engineering: Past, present and future. Journal of Wind Engineering and Industrial Aerodynamics, 179, 1-75.
[13] Huebner, K. H., Dewhirst, D. W., Smith, D. E., & Byrom, T. G. (2008). The finite element method for engineers. John Wiley & Sons.
[14] Crawley, D. B., Hand, J. W., Kummert, M., & Griffith, B. T. (2008). Contrasting the capabilities of building performance simulation programs. Building and Environment, 43(4), 381-392.
[15] Wetter, M., Horsch, T., & Nouidui, T. S. (2011). Functional mockup interface for co-simulation of building energy and control systems. In Proceedings of the IBPSA building simulation conference (Vol. 2011, pp. 1088-1095).
[16] Olgyay, V. (2015). Design with climate: Bioclimatic architectural regionalism. John Wiley & Sons.
[17] de Dear, R. J., & Brager, G. S. (2002). Thermal adaptation in the built environment: A literature review. Energy and Buildings, 34(2), 191-204.
[18] Ehrhardt-Martinez, K., Donnelly, K. A., & Laitner, J. A. (2003). Advanced metering initiatives and residential feedback: A meta-review for the US Department of Energy. American Council for an Energy-Efficient Economy, 1, 2-23.
Be the first to comment